Optimization of time for neural stem cells transplantation for brain stroke in rats
Introduction
According to 2016 statistical data from American Stroke Association, brain stroke is ranked one the major cause of death after cardiovascular pathologies and cancer, and projections show that by 2030, 3.4 million more people aged 18 and above will suffer from stroke that will amount to a staggering 20% increase as compared to 2012 (1). Despite successful pharmacological intervention using thrombolytic therapy, the procedural complications combined with very narrow therapeutic time-window for intervention make it worthwhile for no more than 7% of the patients (2). The situation therefore warrants the development of alternative methods of therapeutic intervention.
Recent advancements in stem cell therapy approach have shifted the focus for the management of stroke from neuroprotection to neurorestoration that seeks to replace the damaged cells in the affected-brain with morpho-functionally competent substitute cells. Encouraging data have been reported in the experimental animal models of ischemic stroke using a distinct selection of stem cells of various origins. Noticeable amongst these are neural stem cells (3,4), bone marrow stem cells (3,5,6), cord blood derived MSCs (7,8), adipose tissue derived (5), embryonic stem cells and their derivatives (9), and induced pluripotent stem cells (iPSCs) (10,11). Nevertheless, ease of availability without moral and ethical strings and differentiation capacity to adopt desired phenotype are vital issues for the search of ideal donor cells for transplantation therapy. Given their association with the nervous system and capability of differentiation into the three neural lineages including neurons, oligodendrocytes and astrocytes, neural stem cells in particular are being considered as near-ideal cells for the treatment of stroke (3).
Experimental animal studies provide pre-clinical evidence regarding the safety and feasibility of the stem cell based reparative approach for ischemic stroke which has been vindicated during the clinical studies (12,13). The beneficial therapeutic outcome from the donor cells is considered as multifactorial that ranges from neurogenesis to the release of bioactive molecules as part of their paracrine activity to support the endogenous repair mechanisms in the damaged area of the brain (14,15). The donor cells repopulate the ischemic area and get integrated into the host circuit besides providing protection benefits to impart functional recovery (3,14,16). Despite encouraging results, multiple variables that are determinants of the success of cell therapy for stroke treatment remain less well-studied. These variables range from cell type selection to route of administration and optimum time duration after stroke to carry out stem cell transplantation to achieve best prognosis. The present study was aimed to assess the optimal time of NSC therapy post ischemic insult. Using rodent model of experimental ischemic stroke by middle-cerebral artery occlusion (MCAO), in vitro cultured NSCs were injected at stipulated time-points ranging from 1 hour to 7 days. The animals after their respective treatment on the stipulated time points were assessed for the neurological outcome, dUTP nick end labeling (TUNEL) assay and also the Caspase 3 activity to identify the apoptosis. Our results highlight the importance of early injection of the stem cells to curtail ischemic tissue injury to the brain during stroke.
Methods
The present study conformed to the Guideline for the Care and Use of Laboratory Animals and all the experimental animal procedures were performed strictly in accordance with protocol approved by Shiraz University of Medical Sciences, Iran. All surgical manipulations were carried out under general anesthesia.
Isolation of NSCs
NSCs were isolated from the ganglion eminences dissected from E14 (14-day-old) embryos of Sprague-Dawley rats using our standard protocol. Briefly, the heads of the embryos were separated and the brain tissue was dissected to separate cortices, midbrain and stria. The dissected tissue was transferred to the NSC culture media DMEM/F12 (Invitrogen Cat #10565018) supplemented with 2% B27 (Gibco Cat #17504044), 1% N2 (Invitrogen Cat #17502048, 10 ng/mL basic fibroblast growth factor (bFGF; Sigma Cat #F0291) and 20 ng/mL epidermal growth factor (EGF; Sigma E9644). The isolated tissues were mechanically dissociated and pipetted for reaching single cells to make a uniform suspension. The cells were seeded at density 50,000 cells/mL in culture dish at 37 °C and 5% CO2. Neurospheres appeared by day 5 (17). For identification of NSCs, immunocytochemistry was performed using antibodies specific for Nestin (Abcam Cat #6142) and CD133 (Millipore; Cat# MAB4399) respectively.
Tri-lineage differentiation of NSCs
Single cell suspension of passage# 4 NSCs was prepared by treatment with 0.05% trypsin (Gibco Cat #25300054). The cells were later cultured on polyornithine coated plates (Sigma Cat #P3655) for 2 days. For induction of tri-lineage neural differentiation, 0.5% fetal bovine serum (FBS) (Gibco Cat #26140079) was added to the NSCs culture medium while concomitantly removing both bFGF and EGF. Three days later, the NSCs were differentiated into neurons, oligodendrocytes and astrocytes. To confirm the differentiation of the NSCs, immunocytochemistry was performed for β-tubulin III (neuron marker), glial fibrillary acidic protein (Gfap; an astrocyte marker) and Oligodendrocyte marker Olig2 as described earlier (18).
Immunocytochemistry for tri-lineages cells markers
Immunostaining of cells for specific markers was essentially carried out according to our standard protocols as described earlier (18). Briefly, the cells were cultured on glass slides and fixed with 4% paraformaldehyde for 20 minutes at 4 °C. The cells were washed ×3 with phosphate buffered saline (PBS) followed by incubation with respective primary antibody in PBS containing 0.3% triton and 5% goat serum, at room temperature for 1 hour. Primary antibodies used included anti tubulin-III (Promega Cat #G7121; 1:2,000), anti-Olig2 antibody (Millipore Cat# AB9610; 1:500) and anti-Gfap (Dako Cytomation Cat #Z0334; 1:500) for neurons, oligodendrocytes and astrocyte detection respectively. The cells were then washed ×3 with PBS and respective incubated with fluorescent-conjugated secondary antibodies for 45 minutes at room temperature. The nuclei were labeled with 4,6-diamino-2-phenylindole dihydrochloride (DAPI; Millipore Cat #S7113, 1:1,000) as described earlier (18). The samples were later fixed and visualized under fluorescence microscope (Olympus BX53 Japan) fitted with camera and software Cell-sens.
Experimental animal model of ischemic stroke and cell transplantation
The rodent experimental model ischemic stroke was developed in young (10–12 week old) male Sprague Dawley rats (n=120) each weighing 250-300 g by MCAO as described earlier (19). All the animals were allowed for free access to food and water before and after the surgical procedure. Briefly, the rats were anesthetized using Isoflurane (induction 5% and maintenance 1%). Following tracheal intubation and ventilation using Small Animal Ventilator (Harvard Model-683, USA), a vertical incision was made in the midline of the neck. The right common carotid, internal carotid and external carotid arteries were exposed and separated from vagus nerve. Two loose sutures were prepared below carotid bifurcation and external carotid was clamped, the silicone-coated nylon suture 4.0 was passed through a little incision in common carotid artery. After 30 minutes, the nylon suture was removed and the sutures were tightened up so that the blood could flow via external carotid artery by removing the clamp.
The animals were divided into 8 groups (n=15 animals/group) for their respective treatment. The sham group (G-1) did not receive any treatment whereas control group (G2) received 200 µL PBS. For experimental groups (EG) of animals, cell transplantation was carried out at 1 hour (EG1), 12 hours (EG2), 1 day (EG3), 3 days (EG4), 5 days (EG5) and 7 days (EG6) after MCAO respectively. For stereotactic injection of NSCs at stipulated time-points after induction of MCAO, the animals were anesthetized with Isoflurane (induction 5% and maintenance 1%) and then fixed to the stereo tactical frame. A total of 200,000 cells suspended in 200 µL PBS were injected into right lateral ventricle at anteroposterior (AP) =−0.12 mm, mediolateral (ML) =1.6 mm and dorso-ventricular (DV) =4.3 mm. The animals were allowed to recover and buprenex (0.1 mg/kg b.i.d) was administered for 24 h to alleviate pain.
Histological studies
After 28 days of their respective treatment, the animals were euthanized with deep anesthesia and perfused with normal saline followed by Paraformaldehyde 4%. The specimens of brain were prepared for cryosections (at a thickness of 10 µm) and then the sections were mounted on silicon pre-coated slides. The specimens were stained with Hematoxylin & Eosin (H&E) to visualize the architecture.
Caspase-3 and TUNEL assays
TUNEL assay was performed to determine the number of apoptotic cells in the damaged area. The sections were stained by using terminal deoxynucleotidyl transferase-mediated (TUNEL) in situ Apoptosis Detection Kit (Chemicon International, Inc., USA).The images were taken by fluorescent microscopy (Olympus BX53, Tokyo, Japan).
Neurological function assessment
The neurological examinations of the animals were performed every 2 days for all rats during 28 days of experiment after their respective treatment. The neurological examination was scored on 0–5 score scale as described earlier (19). The scores criteria was: No neurological deficit (score =0); failure to extend the left forepaw completely to reflect mild focal neurological deficit (score =1); circling to the left to reflect a moderate focal neurological deficit (score =2); falling to the left indicating a sever focal neurological deficit (score =3); no spontaneous walking and decreased level of consciousness (score =4) and death due to brain ischemia (score =5).
Statistical analysis
Data were presented as mean ± SD. For quantitative analysis, data was analyzed with student t-test and one-way ANOVA with post hoc analysis using SPSS 16.00. A value of P<0.05 was considered as statistically significant.
Results
NSCs expansion and characterization
The NSCs were successfully isolated from rat embryos and cultured in vitro under well-defined culture conditions. On day 5 in culture after isolation, NSCs were observed to form neurospheres (Figure 1A). Immunocytochemistry of the isolated neural cells that formed the neurospheres stained positively for the expression of specific markers including Nestin (Figure 1B,C) and CD133 antibody (Figure 1D,E). Removal of growth factors bFGF and EGF and supplementation of the culture medium with 5% FBS promoted trilineage neural differentiation of the NSCs. Immunofluorescence staining using β-tubulin-III antibody (for neuron detection), Gfap (for astrocyte detection) and Olig-2 (for Oligodendrocyte detection) showed that 17.43%±3.02% of the cultured NSCs were positive for β-tubulin-III (Figure 1F) while 70.50%±6.29% of the differentiated cells were positive for Gfap (Figure 1G) and 8.94%±1.32% cells were positive for Olig-2 (Figure 1H).
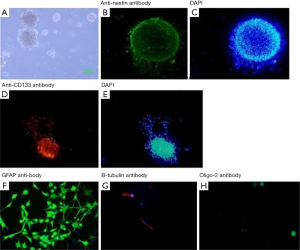
Histological studies
At stipulated time-points, the animals were deeply anesthetized and perfused with normal saline followed by 4% Paraformaldehyde. The cryosection of 10 µm thickness were cut and selected with 1 mm interval and the first one was 1 mm posterior to frontal lobe. The damaged area was determined by ischemic sings containing eosinophilic cytoplasm and pyknotic nuclei. The volume area of sham group was 191.39±12.53 m3 and the group which received NSCs 3 days after ischemia had the least volume of damaged area 68.13±4.93 m3. There was a significant difference between the 3 days after ischemia receiving NSCs and the rest groups Tukey’s multiple comparison test and also all groups’ neurological outcomes had significant differences in comparison to sham group (Figures 2,3). These data confirm that the NSCs transplantation 3 days after stroke could promote histologically improvement of the brain.
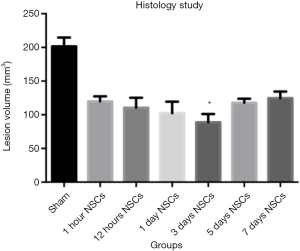
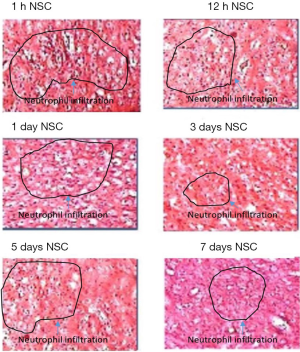
Apoptosis evaluation
Caspase 3 is an integral member of the apoptosis cascade (20). The caspase-3 activity assay in different treatment groups showed least amount of caspase-3 activity in the day 3 treated animal group after stroke as compared to all other groups (P<0.05; Figure 4). These data were duly supported by TUNEL assay which showed that TUNEL positive cells were least in the day 3 treatment animal groups after stroke injury as compared to all other groups (Figure 5). The highest level of TUNEL positive cells was observed in the animals which did not receive NSCs after stroke injury. The number of TUNEL positive cells was 12.92%±2.26% in that group and there is a significant difference between that group and the others (P<0.05). Histological studies after H&E staining showed significant infiltration of inflammatory cells in the ischemic zone which was significantly reduced in the day 3 animal groups as compared to the other treatment groups (Figure 3).
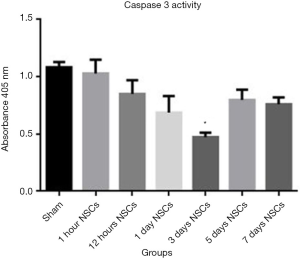
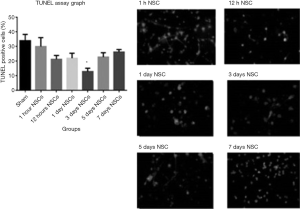
Neurological examination
All of the groups were examined during the experiment for the stipulated time duration of 28 days. The animals in each group completed full length of the experiment and there was no death related with the cell treatment. Neurological examination scores were analyzed with Sidak’s multiple comparison tests. There was significant difference between the mean of the 3 days NSCs injection neurological scores (2.99±0.19) with sham operated group and all the other cell treatment groups (P<0.05 vs. all other treatment groups). Also, there were significant differences (P<0.05) between the sham group and all cell therapy groups (Figure 6).
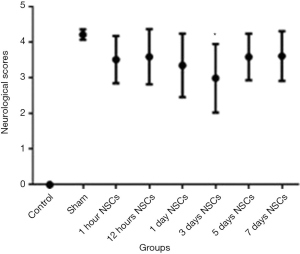
The final goal of every treatment on stroke could be described as enhancing neurological function. According to these data, all cell therapy groups could perform better than sham operated group. In addition, a significant difference is observable in all cell therapy groups which means that different cell therapy protocol could modify the outcome of the treatment. The graph in Figure 6 shows the NSC transplantation 3 days after stroke could be considered as an effective way to optimize the neurological performance.
Discussion
The main findings of our study are that the: (I) NSCs successfully attenuated infarct size expansion and provided best neurological outcome; (II) optimum time for stem cell therapy was 3 days post ischemic stroke; and (III) NSC transplantation prevented host cell apoptosis as one of the possible underlying mechanisms to attenuate infract size expansion. It is noteworthy that attenuated infarct size, reduction in TUNEL positivity and decreased caspase levels corresponded well with day 3 of NSC transplantation.
The brain being a highly perfused organ in the body utilizes more than 20% of the total oxygen and hence the cells therein are exceedingly sensitive to ischemia (21). Subsequent to the ischemia, as the events progress, inflammatory response causes loss of neural cells both in the center of the ischemic zone, mainly by necrosis, as well as in the penumbra, mostly by apoptosis (22,23). During acute phase, elevated levels of the otherwise lowly expressed plethora of molecules such as intercellular adhesion molecule-1 (ICAM-1), P-selectin and matrix metalloproteinases are positively correlated with clinical worsening of stroke patients thus leading to poor prognosis (24,25). Incidentally, polymorphism in MMP-9 gene and the expression of level of MMP-9 has been attributed to the risk of stroke and as a marker for the loss of blood brain barrier, edema and intensity of the inflammatory response following ischemic stroke respectively (26,27). Similarly, the elevated levels of pro-inflammatory cytokines such as tumor necrosis factor-1α (TNF-1α), interleukins (IL) IL-1, IL-6 and IL-10 and the chemokines including macrophage inflammatory protein-1α (MCP-1α) and fractalkine affect higher infarct size following ischemic insult (28,29). The end-result of these molecular events is high level infiltration of the inflammatory cells that renders the ischemic area and its penumbra non-conducive for physiological functioning of the brain cells. Unless a therapeutic intervention prevents these nocuous events, massive death of the brain cells ensues owing to the hostile microenvironment in the ischemic area. The combined effect of the cellular and molecular events also significantly impacts the survival of donor cells at the site of the cell graft that remains a serious concern in regenerative medicine for stroke. NSCs are more prone to succumb to ischemic injury when engrafted for the treatment of ischemic stroke. As little as 0.09% survival rate of the donor cells have been reported in the experimental model of ischemic stroke (30). Although the transplanted cells that survived the initial surge of cell death manage to enter cell cycle, the rate of proliferation was meager and only 0.23% cells were observed in the center and peri-infarct regions on day 10 after intravenous delivery (30). Various strategies have been adopted to enhance the donor cells survival post-engraftment including ischemic preconditioning, pre-treatment with pro-survival pharmacological compounds, growth factors, cytokines and by genetic modification (31-34). Additionally, genetic manipulation of stem cells with hypoxia induced microRNAs (miRS) in general and miR-210 in particular have shown encouraging results in terms of improved cell survival (35). Besides other factors, time of cell engraftment after ischemic injury is considered as an important determinant of donor cell survival. If the cells are transplanted too early during the acute phase of ischemia, the cascade of inflammatory response turns the host tissue environment unfavorable for their survival. On the contrary, if cell transplantation is deferred until the inflammatory response is subsided; it would not be possible for the donor cells to revert the scarring process that would have set-in by that time. Furthermore, brain tissue plasticity would be diminished and apoptosis in second injury could make neural regeneration harder than subacute time. Hence, the time of cellular intervention post ischemic insult is integral to the accomplishment of the desired prognosis. Although methodical and in-depth studies to ascertain optimal intermission between stroke to cell transplantation are still wanting in the literature, various research groups have performed cell transplantation from hours to weeks after ischemic stroke (36,37). Our study was aimed to ascertain the optimal time for cell therapy after stroke in terms of attenuation of cell apoptosis in the host tissue and overall improved neurological performance. We observed that the rate of TUNEL positive cells was significantly higher in the earlier time-points of NSC treatment animal group as compared with the day 3 treatment animal groups. These observations were supported by the attenuated brain lesion volume in day 3 treatment group of animals as compared to the other treatment groups. It would have been interesting to determine the rate of donor cell survival and discriminate the TUNEL positive cells as host and the donor cells which are the two main limitations of our study. Our data is compatible with the previously published studies which demonstrated that the optimum time for intervention with NSCs was 3 days after ischemic stroke and it is related to diminished inflammatory response after the acute phase and the peak of post-ischemic apoptotic surge (29). A recent study involving intra-arterial delivery of mesenchymal stem cells in a rodent model of MCAO showed that time of cellular intervention drastically impacted the donor cell distribution and functional recovery in the experimental group (38). The authors observed motor functional recovery in the animals which received cell transplantation therapy on day 4 as compared to those treated on day 1 and day 7 after experimental ischemic stroke. Moreover, the rate of cell transplantation related mortality amongst the day 1 (10.1%) and day 4 (19.2%) treatment groups was significantly low as compared to the day 7 treated animals (30.8%). The same group of researchers reported day 3 as the optimal time for intra-parenchymal transplantation of NSCs (15). In both the studies, the beneficial effect of cell transplantation was attributed to multiple factors including the release of trophic factors, especially vascular endothelial growth factor (VEGF) and angiogenesis besides timing of cell injection. Paracrine release of neuroprotective, angiogenic and pro-survival trophic factors has also been reported by many other research groups as central to the therapeutic benefits of cell transplantation therapy for stroke albeit with variation in the expression profile of the trophic factors being specific to the cell type used engraftment (8,9,15,39). The bioactive molecules released by the transplanted cells not only promote their own survival but also participate in the endogenous repair mechanisms by augmenting survival of the host cells in the vicinity. These cellular events lead to attenuated infarct size expansion. Although neuronal differentiation of the transplanted cells has also been reported but the rate of cell differentiation is insufficient to justify its serious contribution as a major contributing factor to the overall functional benefits of cell therapy. Therefore, besides choice of ideal donor cell type and appropriate pro-neural differentiation cues, pro-survival strategies would be pivotal to enhance the probability and rate of differentiation of the donor cells. For that matter, timing of cell transplantation would be a fundamental consideration to ensure that the transplanted cells escape the primary inflammatory response due to ischemia.
In conclusion, our data shows that day 3 after ischemic stroke is optimal for cellular intervention in terms of reduced apoptosis and quelling infarction size expansion and preservation of neurological functions. However, more systematic experimental studies would be required wherein donor cell survival and mechanistic molecular insight into the beneficial outcome after cell therapy should be carried out to define parameters such as ideal cell type, route of administration and time of cell delivery before routine application in the clinics.
Acknowledgements
None.
Footnote
Conflicts of Interest: The author has no conflicts of interest to declare.
Ethical Statement: The study was approved by Institutional Ethics Committee of Shiraz University of Medical Sciences, Iran (No. 7643).
References
- Writing Group Members, Mozaffarian D, Benjamin EJ, et al. Heart Disease and Stroke Statistics-2016 Update: A Report From the American Heart Association. Circulation 2016;133:e38-360. [Crossref] [PubMed]
- Wardlaw JM, Murray V, Berge E, et al. Recombinant tissue plasminogen activator for acute ischaemic stroke: an updated systematic review and meta-analysis. Lancet 2012;379:2364-72. [Crossref] [PubMed]
- van Velthoven CT, Kavelaars A, van Bel F, et al. Repeated mesenchymal stem cell treatment after neonatal hypoxia-ischemia has distinct effects on formation and maturation of new neurons and oligodendrocytes leading to restoration of damage, corticospinal motor tract activity, and sensorimotor function. J Neurosci 2010;30:9603-11. [Crossref] [PubMed]
- Hosseini SM, Farahmandnia M, Kazemi S, et al. A Novel Cell Therapy Method for Recovering after Brain Stroke in Rats. Int J Stem Cells 2015;8:191-9. [Crossref] [PubMed]
- Ikegame Y, Yamashita K, Hayashi S, et al. Comparison of mesenchymal stem cells from adipose tissue and bone marrow for ischemic stroke therapy. Cytotherapy 2011;13:675-85. [Crossref] [PubMed]
- Hosseini SM, Samimi N, Farahmandnia M, et al. The Preventive Effects of Neural Stem Cells and Mesenchymal Stem Cells Intra-ventricular Injection on Brain Stroke in Rats. N Am J Med Sci 2015;7:390-6. [Crossref] [PubMed]
- Chien CC, Yen BL, Lee FK, et al. In vitro differentiation of human placenta-derived multipotent cells into hepatocyte-like cells. Stem Cells 2006;24:1759-68. [Crossref] [PubMed]
- Lin YC, Ko TL, Shih YH, et al. Human umbilical mesenchymal stem cells promote recovery after ischemic stroke. Stroke 2011;42:2045-53. [Crossref] [PubMed]
- Liu YP, Seçkin H, Izci Y, et al. Neuroprotective effects of mesenchymal stem cells derived from human embryonic stem cells in transient focal cerebral ischemia in rats. J Cereb Blood Flow Metab 2009;29:780-91. [Crossref] [PubMed]
- Mohamad O, Drury-Stewart D, Song M, et al. Vector-free and transgene-free human iPS cells differentiate into functional neurons and enhance functional recovery after ischemic stroke in mice. PLoS One 2013;8:e64160. [Crossref] [PubMed]
- Yuan T, Liao W, Feng NH, et al. Human induced pluripotent stem cell-derived neural stem cells survive, migrate, differentiate, and improve neurologic function in a rat model of middle cerebral artery occlusion. Stem Cell Res Ther 2013;4:73. [Crossref] [PubMed]
- Hess DC, Sila CA, Furlan AJ, et al. A double-blind placebo-controlled clinical evaluation of MultiStem for the treatment of ischemic stroke. Int J Stroke 2014;9:381-6. [Crossref] [PubMed]
- Qiao LY, Huang FJ, Zhao M, et al. A two-year follow-up study of cotransplantation with neural stem/progenitor cells and mesenchymal stromal cells in ischemic stroke patients. Cell Transplant 2014;23 Suppl 1:S65-72. [Crossref] [PubMed]
- Bühnemann C, Scholz A, Bernreuther C, et al. Neuronal differentiation of transplanted embryonic stem cell-derived precursors in stroke lesions of adult rats. Brain 2006;129:3238-48. [Crossref] [PubMed]
- Horie N, Pereira MP, Niizuma K, et al. Transplanted stem cell-secreted vascular endothelial growth factor effects poststroke recovery, inflammation, and vascular repair. Stem Cells 2011;29:274-85. [Crossref] [PubMed]
- Dai J, Li SQ, Qiu YM, et al. Migration of neural stem cells to ischemic brain regions in ischemic stroke in rats. Neurosci Lett 2013;552:124-8. [Crossref] [PubMed]
- Mattsson B, Sørensen JC, Zimmer J, et al. Neural grafting to experimental neocortical infarcts improves behavioral outcome and reduces thalamic atrophy in rats housed in enriched but not in standard environments. Stroke 1997;28:1225-31; discussion 1231-2. [Crossref] [PubMed]
- Hosseini SM, Talaei-Khozani T, Sani M, et al. Differentiation of human breast-milk stem cells to neural stem cells and neurons. Neurol Res Int 2014;2014:807896.
- Koizumi J, Yoshida Y, Nakazawa T, et al. Experimental studies of ischemic brain edema. I. A new experimental model of cerebral embolism in rats in which recirculation can be introduced in the ischemic area. Jpn J Stroke 1986;8:1-8. [Crossref]
- Fernandes-Alnemri T, Litwack G, Alnemri ES. CPP32, a novel human apoptotic protein with homology to Caenorhabditis elegans cell death protein Ced-3 and mammalian interleukin-1 beta-converting enzyme. J Biol Chem 1994;269:30761-4. [PubMed]
- Clarke DD, Sokoloff L. Circulation and energy metabolism of the brain. In: Siegel G, Agrano BV, Albers RW, et al. editors. Basic Neurochemistry. New York: Raven Press, 1989: 565-90.
- Lipton P. Ischemic cell death in brain neurons. Physiol Rev 1999;79:1431-568. [PubMed]
- Yuan J, Lipinski M, Degterev A. Diversity in the mechanisms of neuronal cell death. Neuron 2003;40:401-13. [Crossref] [PubMed]
- Frijns CJ, Kappelle LJ. Inflammatory cell adhesion molecules in ischemic cerebrovascular disease. Stroke 2002;33:2115-22. [Crossref] [PubMed]
- Jordán J, Segura T, Brea D, et al. Inflammation as therapeutic objective in stroke. Curr Pharm Des 2008;14:3549-64. [Crossref] [PubMed]
- Buraczynska K, Kurzepa J, Ksiazek A, et al. Matrix Metalloproteinase-9 (MMP-9) Gene Polymorphism in Stroke Patients. Neuromolecular Med 2015;17:385-90. [Crossref] [PubMed]
- Ramos-Fernandez M, Bellolio MF, Stead LG. Matrix metalloproteinase-9 as a marker for acute ischemic stroke: a systematic review. J Stroke Cerebrovasc Dis 2011;20:47-54. [Crossref] [PubMed]
- Lakhan SE, Kirchgessner A, Hofer M. Inflammatory mechanisms in ischemic stroke: therapeutic approaches. J Transl Med 2009;7:97. [Crossref] [PubMed]
- Hosseini SM, Farahmandnia M, Razi Z, et al. Combination cell therapy with mesenchymal stem cells and neural stem cells for brain stroke in rats. Int J Stem Cells 2015;8:99-105. [Crossref] [PubMed]
- Bacigaluppi M, Pluchino S, Peruzzotti-Jametti L, et al. Delayed post-ischaemic neuroprotection following systemic neural stem cell transplantation involves multiple mechanisms. Brain 2009;132:2239-51. [Crossref] [PubMed]
- Kim HW, Haider HK, Jiang S, et al. Ischemic preconditioning augments survival of stem cells via miR-210 expression by targeting caspase-8-associated protein 2. J Biol Chem 2009;284:33161-8. [Crossref] [PubMed]
- Niagara MI, Haider HKh, Jiang S, et al. Pharmacologically preconditioned skeletal myoblasts are resistant to oxidative stress and promote angiomyogenesis via release of paracrine factors in the infarcted heart. Circ Res 2007;100:545-55. [Crossref] [PubMed]
- Ye L, Haider HKh, Tan R, et al. Transplantation of nanoparticle transfected skeletal myoblasts overexpressing vascular endothelial growth factor-165 for cardiac repair. Circulation 2007;116:I113-20. [Crossref] [PubMed]
- Elmadbouh I, Haider HKh, Ashraf M, et al. Preconditioning of Human Skeletal Myoblast with Stromal Cell-derived Factor-1α Promotes Cytoprotective Effects against Oxidative and Anoxic Stress. Int J Stem Cells 2011;4:50-60. [Crossref] [PubMed]
- Kim HW, Jiang S, Ashraf M, et al. Stem cell-based delivery of Hypoxamir-210 to the infarcted heart: implications on stem cell survival and preservation of infarcted heart function. J Mol Med (Berl) 2012;90:997-1010. [Crossref] [PubMed]
- Darsalia V, Allison SJ, Cusulin C, et al. Cell number and timing of transplantation determine survival of human neural stem cell grafts in stroke-damaged rat brain. J Cereb Blood Flow Metab 2011;31:235-42. [Crossref] [PubMed]
- Rosenblum S, Wang N, Smith TN, et al. Timing of intra-arterial neural stem cell transplantation after hypoxia-ischemia influences cell engraftment, survival, and differentiation. Stroke 2012;43:1624-31. [Crossref] [PubMed]
- Ishizaka S, Horie N, Satoh K, et al. Intra-arterial cell transplantation provides timing-dependent cell distribution and functional recovery after stroke. Stroke 2013;44:720-6. [Crossref] [PubMed]
- Kurozumi K, Nakamura K, Tamiya T, et al. Mesenchymal stem cells that produce neurotrophic factors reduce ischemic damage in the rat middle cerebral artery occlusion model. Mol Ther 2005;11:96-104. [Crossref] [PubMed]
Cite this article as: Ziaee SM, Tabeshmehr P, Haider KH, Farrokhi M, Shariat A, Amiri A, Hosseini SM. Optimization of time for neural stem cells transplantation for brain stroke in rats. Stem Cell Investig 2017;4:29.