An ex vivo human placental vessel perfusion method to study mesenchymal stem/stromal cell migration
Introduction
Mesenchymal stem/stromal cells (MSCs) are well-established, important players in tissue repair and regeneration. The conventional concept of MSC-based tissue repair is that MSCs are mobilised to their target by chemoattractant signals released by the damaged tissue. Regardless of their source, MSCs respond to the inflammatory signal(s) by entering the blood stream, migrating to the target area, crossing the endothelial barrier and homing in to the damaged tissue (1,2). MSCs then initiate repair by differentiating into the appropriate cell type to replace the damaged tissue, or by releasing paracrine factors that act on resident cells, or by modulating inflammatory processes (3-6). Therefore, MSCs must be able to efficiently migrate to, and interact with, the microenvironment at the site of injury. This process is not fully understood in humans. The aim of this study therefore, was to develop an ex vivo human placental vessel perfusion method to examine MSC migration from the circulation and into tissue. This will provide a better understanding of MSC transendothelial migration and engraftment into target tissue, in a setting that more closely represents an in vivo state.
Perfusion is a technique where a fluid is injected into a blood vessel in order to reach an organ or a tissue. The placental perfusion system was first developed and described by Panigel in 1962 (7), then later modified by Schneider and Huch in 1985 (8), and other research groups, including our own (9). Perfusion of the human placenta is one of the most useful methods to examine the transplacental transfer of chemical substances and drugs through the maternal-fetal interface (10-13). The placental perfusion model has also been used to investigate the transplacental transfer of antigens, such as the blood stage malaria antigen (14), or to study the transfer of immune cells from the mother to the fetus (15), and the invasion of leukaemia cells into fetal tissues (16).
Stem cell researchers have utilized the perfusion technique to study MSC transmigration and homing in various organs. For example, Nazarov et al. [2012] perfused MSCs into an ex vivo human acute lung injury model to assess their therapeutic effect (17). Yet the majority of other studies involve perfusion of human MSCs into animal organs, most commonly murine hearts (18,19). This is because the ability to perfuse human organs, ex vivo, is limited by the availability of organs, and the difficulty in maintaining the physiology of the perfused organ in an ex vivo sitting for the duration of the experiment. The term human placenta remains largely unexploited for investigating MSC transendothelial migration and engraftment. Unlike other human organs, the term human placenta is abundant and readily available but more importantly, the ex vivo perfused placenta can be maintained in its physiological state for up to several days.
Here, we employ a term human placenta ex vivo perfusion method, where placental vessels are perfused ex vivo with a medium containing MSCs that have been stained with live-cell fluorescent dyes. We employed placental CMSC29 and DMSC23 cell lines, which were derived by hTERT transformation of primary, term, chorionic and decidua basalis MSCs respectively (see below). Both cell lines were verified to maintain the MSC phenotype and functions, including the ability to migrate. With this method, MSCs were observed to migrate from the vessel lumen into the vessel wall and cross the endothelial cell barrier.
Methods
Tissue collection
Placentae for this project were collected with approval from the Royal Women’s Hospital (RWH) Human Research and Ethics Committees. All placentae were obtained following informed, written patient consent at the RWH, Parkville, Australia. The collected placentae were from clinically uncomplicated, healthy pregnancies and had no obvious macroscopic defects.
Cell culture
The CMSC29 and DMSC23 cell lines were used in this study as representatives of chorionic and decidual MSC types (CMSCs and DMSCs). CMSC29 and DMSC23 were created at the Pregnancy Research Centre (PRC)-RWH laboratory from primary, term, chorionic and decidua basalis MSCs respectively, by hTERT transformation (20). Both cell lines have been verified to maintain MSC characteristics and functions, including migration capacity (20). CMSC29 cells were cultured in AmnioMAX™ C-100 Basal Medium supplemented with AmnioMAX™ C-100 Supplement [(10:1, v/v), Life Technologies, Carlsbad, CA, USA] (21). DMSC23 cells were cultured in MesenCult™ Proliferation Kit (Human) [MesenCult™ MSC Basal Medium and Mesenchymal Stem Cell Stimulatory Supplements (10:1, v/v), STEMCELL Technologies, Vancouver, Canada]. Cells were kept in a humidified incubator at 37 °C, 5% CO2 and 95% room air.
Optimizing live-cell fluorescent labelling for CMSC29 and DMSC23 cells
Prior to perfusing, CMSC29 and DMSC23 cells were labelled with live-cell fluorescent dyes. This was done to detect the CMSC29 or DMSC23 cells in the perfused vessel sections. Cell trackerTM Orange (CMTMR) and Cell trackerTM Green (CMFDA) live-cell florescence dyes (Life Technologies), were used to label CMSC29 and DMSC23 cells respectively. According to the manufacturer’s instructions, the recommended concentration for long-term staining (i.e., >3 days) of rapidly dividing cells was 5–25 µM dye, incubated for 15–45 minutes. However, a report noted that increasing the CMTMR loading time for up to 2 hours leads to better dye retention by the cells with no impact on viability (22). Therefore, to optimise the CMTMR and CMFDA concentration and incubation time for CMSC29 and DMSC23 labelling, five dye concentrations and five incubation times were tested. Cells suspended in supplemented medium were seeded into 6-well plates and left to adhere and grow overnight. Five wells per plate were seeded, one for each concentration tested (15, 20, 25, 30 and 35 µM). Five plates in total were used, one for each incubation time tested (0.5, 1, 1.5, 2 and 2.5 hours).
To label the cells, cell culture medium was removed from the wells and pre-warmed dye working solution was added at 15, 20, 25, 30 or 35 µM. The cells were incubated for 0.5, 1, 1.5, 2 or 2.5 hours, under cell growth conditions. The dye working solution was then replaced with supplemented medium and cells were incubated for another 30 minutes under cell growth conditions. Finally, the medium was removed, and the cells were washed with phosphate buffer saline (PBS) before fresh supplemented medium was added. At day 1 and day 3 after cell labelling, the fluorescence intensity was examined by measuring the pixel intensities with an Olympus IX2 inverted microscope (Olympus Shinjuku, Tokyo, Japan) equipped with CellR imaging software (Olympus). To assess whether the used cell tracker concentrations affected cell viability, 3×105 cells were seeded per tube and stained using the manufacturer’s “cells in suspension” staining protocol. Then, a viability count was carried out on the stained cell suspension and compared to the control.
Establishment of the ex vivo placental vessel perfusion method
Following delivery at term, the placenta was kept at 4 °C and transported to the laboratory within 20 minutes. The placenta was then assessed for any macroscopic abnormalities and perfused within two hours. The placenta was placed in the upper compartment of a dual compartment perfusion bath. The lower compartment was pre-filled with water and maintained at 37 °C (Figure 1). The blood vessel (vein or artery) chosen for perfusion was 2–4 mm in diameter and located in the central area of the chorionic plate, in a close proximity to the umbilical cord. The perfused vessel was isolated from the placental circulation by applying two tight stiches approximately 4 cm apart and the isolated area of the vessel was cannulated. The cannulas were stabilized by applying another two stiches leaving around 1–1.5 cm of vessel length between the inflow cannula and the outflow cannula. Following cannulation, the placenta was submerged in warm saline and the cannulated vessel was washed for 1 hour. Washing was done with warm Krebs-Henseleit buffer balanced salt solution (23), saturated with 5% O2 and 5% CO2 in N2. Perfusion was at a constant pressure of 5 ml/min using a peristaltic pump (Thermo Fisher Scientific, catalogue No. 7554-20).
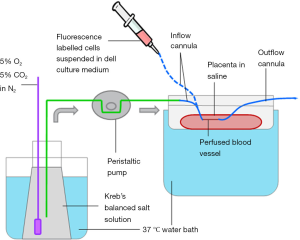
After the first wash was completed, fluorescently labelled MSCs were suspended in cell culture medium (Av.1.5×106 cells/ml in a volume of 3 mL) and perfused into the vessel. The labelled MSCs were injected into the perfused vessel by disconnecting the inflow cannula from the peristaltic pump circuit, and connecting the cannula to a syringe containing the cell suspension. After the cells were perfused into the vessel, the vessel was dissected from the placenta with the stabilized cannulas still attached. Then, the dissected vessel was placed in a Petri dish filled with saline and incubated at cell growth conditions (37 °C, 5% CO2 and 95% room air) for 3 hours up to 4 days. Following incubation, the vessel was taken out of the incubator and the perfusate was drained and the lumen of the vessel was washed thoroughly with cell culture medium using the attached cannulas and a syringe.
Finally, the perfused vessel was transferred into a foil cylinder filled with Jung Tissue Freezing Medium™ Jung (Leica Biosystems, Nuloch, Germany), and snap frozen in liquid nitrogen. The frozen vessel was either sectioned immediately or stored at −80 °C until sectioning. For each perfused vessel, the block was sectioned into 20 µm × 5 µm cross sections using a cryostat (Leica CM1850 UV, Leica Bioscience). The 20 sections collected were obtained from different segments across the approximately 1 cm perfused vessel. Prior to examination, the sections were fixed with 70% ice cold ethanol [(v/v) in Milli-Q water] and immunofluorescence detection of the endothelium was carried out. The endothelium was detected with a conjugated anti-vWF antibody as described below. Cell nuclei were detected by using mounting medium for fluorescence containing DAPI nuclear stain (VECTASHIELD®, Vector Labs, Burlingame, CA, USA). Images were taken using an Olympus Provis AX-70 fluorescence microscope (Olympus) equipped with AxioVision 40 version 4.8.2.0 software (Carl Zeiss AG, Oberkochen, Germany). The criterion for migration was the detection of labelled CMSC29 or DMSC23 that had crossed the endothelial barrier.
Endothelium labelling with anti-von Willebrand factor (vWF) antibody
The endothelium of the perfused sectioned vessels was labelled with fluorescein-conjugated anti-vWF antibodies. This was done to identify the endothelial barrier and determine whether the fluorescently labelled cells had crossed this barrier. Two anti-vWF antibodies were used in this study. Antibody concentrations and incubation times were optimized for 5 µm placental vessel sections, based on qualitative assessment of fluorescence signal intensity under a microscope. The first was a FITC-conjugated sheep antibody to human vWF (Meridian Bioscience, Cincinnati, OH, USA). Sections were fixed with 70% ethanol [(v/v) in Milli-Q water] before blocking with 5% skim milk [(w/v) in PBS] for 1 hour at room temperature. After blocking, the sections were washed with PBS. Then, the anti-vWF antibody was added at 1:50 dilution [(v/v) in PBS], and incubated for 3 hours at 4 °C in a humidified chamber. The second vWF-antibody used in this study was a rabbit antibody to human vWF (Thermo Fisher Scientific, Waltham, Ma, USA), together with a Cy5-conjugated goat antibody to rabbit IgG (Abcam PLC, Cambridge, UK). The sections were first fixed, blocked and washed as described. Then, the rabbit antibody to human vWF was added at 1:50 dilution [(v/v) in PBS], and incubated overnight (at least 18 hours) at 4 °C in a humidified chamber. Next, the tissue sections were washed with PBS and conjugated goat antibody to rabbit IgG diluted at 1:100 [(v/v) in PBS], was added to the sections and incubated for 3 hours at 4 °C in a humidified chamber.
Results
Optimizing live-cell fluorescent labelling for CMSC29 and DMSC23 cells
The fluorescence intensity of CMTMR labelled CMSC29 cells and CMFDA labelled DMSC23 cells continued to increase up to 30 µM of dye concentration, before reaching a plateau (Figure 2A,B). For CMTMR, most of the tested concentrations gave the highest pixel intensity values following 2 hours of incubation. Whereas for CMFDA, most of the tested concentrations gave the highest pixel intensity values following 1.5 hours of incubation (data not shown). Comparing fluorescence intensity following one day and three days of labelling, it appeared that the fluorescence intensity was qualitatively higher at day three than it was at day one for CMTMR labelled CMSC29 cells, and qualitatively lower at day three than it was at day one for CMFDA labelled DMSC23 cells (Figure 2A,B). At all tested concentrations, there was no obvious change in the characteristic spindle-shaped MSC morphology and both CMSC29- and DMSC23-labelled cells were able to proliferate and achieve confluency (Figure 2C,D). The mean percentages of viable, labelled CMSC29 at 30 µM CMTMR for 2 hours was 88.3%±5.8% (SEM). The mean percentages of viable, unlabelled control CMSC29 cells was 81.7%±1.7% (SEM) (n=3). This indicates that using the Cell trackerTM Probes at a concentration of 30 µM, incubated for up to 2 hours, give high fluorescence intensity without affecting cell viability.
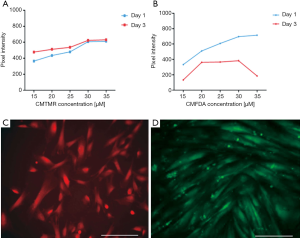
Establishment of the ex vivo placental vessel perfusion method
Twelve placental vessel perfusions were successfully completed. In eight of the twelve perfused vessels, qualitative assessment of immunofluorescence in cross sections (n=20, 5 µm sections/vessel) revealed labelled CMSC29 cells crossed the endothelium barrier (Figure 3). Preliminary results from one of the vessels (n=20, 5 µm sections) showed an average of 1.05 cells/5 µm section had crossed the endothelial barrier (i.e., 210 CMSC29 cells/1 cm of vessel, constituting around 0.2% of perfused cells). Fluorescent cells were also seen protruding between the endothelial cells (Figure 4A,B). Other fluorescent cells were seen aggregated or attached to the luminal side of the endothelial lining (Figure 4C,D). These fluorescent cells are believed to be adhered to the endothelium, since these cells were not removed from the luminal wall despite extensive washing of the vessel prior to sectioning. A negative control was included, which consisted of non-labelled CMSC29 cells perfused into a placental vessel and then incubated. After washing to remove unattached cells, sections were prepared, stained with DAPI and examined for any fluorescence. In all 20 sections examined, no red-orange fluorescence was detected (data not shown).
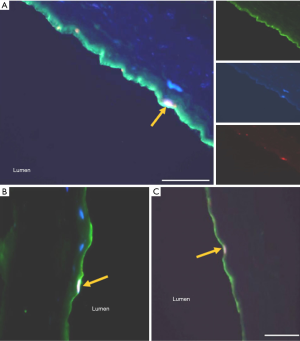
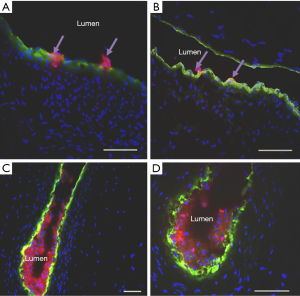
Perfusion of a mixture of labelled DMSC23 and CMSC29 cells into vessels
Labelled CMSC29 (CMTMR-orange) cells and labelled DMSC23 (CMFDA-green) cells were mixed in equal numbers and then perfused into placental vessels. This was done to determine whether diverse placenta-derived MSCs would behave differently in the same perfused vessel. For example, one MSC type may cross the endothelial barrier more efficiently in the same microenvironment within the perfusion vessel. Qualitative assessment of immunofluorescence in sections from two repeats revealed that both labelled CMSC29 and DMSC23 cells crossed the endothelial barrier (Figure 5) but there was no qualitative difference in the number of MSCs of each type that transmigrated across the endothelial barrier.
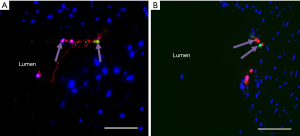
Discussion
During the development and optimization of this ex vivo perfusion system, transformed MSC cell lines were used to remove the confounding variable of patient-to-patient variation in primary MSC preparations. Nevertheless, other variables were observed between experiments; which included the type of the vessel perfused i.e. vein or artery, and the incubation time of cells inside the perfused vessel, which ranged between 3 hours up to 4 days. The alteration in the incubation time in different repeats was to assess the effect of different incubation periods on the efficiency of cell migration. Regardless of the type of vessel and incubation time, in eight out of twelve completed perfusions, there was evidence of successful cell migration through the perfused vessel endothelium. With regard to the 4 vessels where no fluorescent cells were observed, it could be that the 20 µm × 5 µm sections obtained from these vessels were not sufficient to detect any of the fluorescent cells since they represent only 1% per cm of perfused vessel area.
The mechanisms involved in this trans-endothelial movement are not yet fully understood. MSCs are assumed to utilize the same key mechanism employed by leukocyte recruitment to inflamed or injured areas (24). Indeed, in some of the examined sections, perfused cells were observed adhering to the luminal side of the endothelium, or protruding in between endothelial cells, which resembled the behaviour of leukocytes in blood vessels (Figure 4). Another interesting finding was that MSCs that had completely crossed the endothelial barrier, were always found to be in close proximity to the endothelial cells and were not found anywhere in the stroma or within the smooth muscle layer (Figure 3). The close proximity of migrated cells to the endothelium coincides with the reported MSC niche in the placenta. Both CMSCs in chorionic villous tissues, and DMSCs in the decidua, reside in a vascular niche (25,26). In this perfusion system, migrating CMSC29 and DMSC23 cells crossed the endothelial barrier within as little as 3 hours and remained in a location consistent with the MSC niche adjacent to endothelial cells even after 4 days of incubation. This contrasts with the results seen with other cells, for example, when labelled maternal lymphocytes were perfused into the maternal-side of the placenta, the lymphocytes crossed the endothelial barrier, migrated through the stroma and infiltrated the fetal villous structures (15). A possible explanation for the migration pattern of cells in this perfusion system is that there was no damage to the perfused tissue, and thus, the MSCs did not receive the appropriate damage signal to promote their movement into the tissue stroma, and instead remained in their niche. Since MSCs are reported to have a similar migration pattern to leukocytes, we expect that induction of damage to the endothelial layer will increase MSC migration in this perfusion model. Endothelial cell damage triggers the release of proinflammatory cytokines, which in turn elevate the expression of molecules involved in the transendothelial movement across the targeted endothelial site (27-29). These molecules include selectins and integrins, which are key players in the leukocyte adhesion cascade, and these factors are also involved in MSC transendothelial movement (19,30). The endothelial layer could be activated in this model using several different methods. For example, lipopolysaccharide (LPS) is a commonly used agent to activate endothelial cells to mimic the inflamed microenvironment (31). The perfused vessels can be perfused with LPS prior to cell introduction to determine whether cells preferentially migrate through the activated endothelium compared with the intact endothelium. Another approach is to perfuse the MSCs into vessels under hypoxic conditions to mimic the tissue microenvironment found in various pathologies.
Finally, both CMSC29 and DMSC23 labelled cells were perfused to determine whether diverse placenta-derived MSCs would behave differently in the same perfused vessel. Both cell types were detected crossing the endothelial cell barrier with no qualitative difference. Other combinations of MSC types (e.g., placental MSC versus adipose tissue MSCs or bone marrow MSCs), or dissimilar stem cells such as embryonic stem cells or induced pluripotent stem cells, could be investigated in the future.
In conclusion, the data from this study indicate that the human placental ex vivo vessel perfusion method could be used to assess human MSC migration into human blood vessels. Moreover, the method can be used to assess the migration of MSCs in an environment that could mimic different pathological conditions, or it could be used to examine and compare the migration of more than one MSC sub-type in the same microenvironment within the blood vessel. Further studies are needed to determine why MSCs that migrate across the vessel wall remain in close proximity to the endothelium, within the vascular niche of MSCs, and do not migrate into the surrounding tissue.
Acknowledgements
We are thankful for the mothers who donated their placenta towards this study, and the clinical research midwives; Sue Nisbet and Sue Duggan at the RWH for placentae collection. We would also like to acknowledge Dr. Neil Gude for his technical guidance during this study.
Funding: The work was supported in part by King Abdulla International Medical Research Center (Grant No. RC08/114). Batla Al-Sowayan was supported by a dual scholarship from the Ministry of National Guard Health Affairs Scholarship Program, Ministry of National Guard-Kingdom of Saudi Arabia and the King Abdullah Scholarship Program, Ministry of Higher Education-Kingdom of Saudi Arabia.
Footnote
Conflicts of Interest: The authors have no conflicts of interest to declare.
Ethical Statement: The study was approved by the Human Research Ethics Committee of the Royal Women’s Hospital, Parkville, Australia (No. 05/07). All placentae were obtained following informed, written patient consent at the RWH, Parkville, Australia.
References
- Squillaro T, Peluso G, Galderisi U. Clinical Trials With Mesenchymal Stem Cells: An Update. Cell Transplant 2016;25:829-48. [Crossref] [PubMed]
- Huber-Lang M, Wiegner R, Lampl L, et al. Mesenchymal Stem Cells after Polytrauma: Actor and Target. Stem Cells Int 2016;2016:6289825. [Crossref] [PubMed]
- Xie J, Peng C, Zhao Q, et al. Osteogenic differentiation and bone regeneration of iPSC-MSCs supported by a biomimetic nanofibrous scaffold. Acta Biomater 2016;29:365-79. [Crossref] [PubMed]
- Teixeira GQ, Pereira CL, Ferreira JR, et al. Immunomodulation of human mesenchymal stem/stromal cells in intervertebral disc degeneration: insights from a proinflammatory/degenerative ex vivo model. Spine (Phila Pa 1976) 2018;43:E673-82. [Crossref] [PubMed]
- Gao F, Chiu SM, Motan DA, et al. Mesenchymal stem cells and immunomodulation: current status and future prospects. Cell Death Dis 2016;7:e2062. [Crossref] [PubMed]
- Zhang S, Chuah SJ, Lai RC, et al. MSC exosomes mediate cartilage repair by enhancing proliferation, attenuating apoptosis and modulating immune reactivity. Biomaterials 2018;156:16-27. [Crossref] [PubMed]
- Panigel M. Placental perfusion experiments. American Journal of Obstetrics and Gynecology 1962;84:1664-72. [Crossref]
- Schneider H, Huch A. Dual in vitro perfusion of an isolated lobe of human placenta: method and instrumentation. Contrib Gynecol Obstet 1985;13:40-7. [PubMed]
- Osmond DT, King RG, Brennecke SP, et al. Placental glucose transport and utilisation is altered at term in insulin-treated, gestational-diabetic patients. Diabetologia 2001;44:1133-9. [Crossref] [PubMed]
- Earhart AD, Patrikeeva S, Wang X, et al. Transplacental transfer and metabolism of bupropion. J Matern Fetal Neonatal Med 2010;23:409-16. [Crossref] [PubMed]
- Berveiller P, Mir O, Vinot C, et al. Transplacental transfer of oseltamivir and its metabolite using the human perfused placental cotyledon model. Am J Obstet Gynecol 2012;206:92 e1-6.
- Porter C, Armstrong-Fisher S, Kopotsha T, et al. Certolizumab pegol does not bind the neonatal Fc receptor (FcRn): Consequences for FcRn-mediated in vitro transcytosis and ex vivo human placental transfer. J Reprod Immunol 2016;116:7-12. [Crossref] [PubMed]
- Smith JA, Gaikwad A, Mosley S, et al. Utilization of an ex vivo human placental perfusion model to predict potential fetal exposure to carboplatin during pregnancy. Am J Obstet Gynecol 2014;210:275.e1-9. [Crossref] [PubMed]
- May K, Grube M, Malhotra I, et al. Antibody-dependent transplacental transfer of malaria blood-stage antigen using a human ex vivo placental perfusion model. PLoS One 2009;4:e7986. [Crossref] [PubMed]
- Heinzelmann J, Enke U, Seyfarth L, et al. Development of a human model to study homing behavior of immune cells into decidua and placental villi under ex vivo conditions. Am J Reprod Immunol 2009;61:19-25. [Crossref] [PubMed]
- Schamberger S, Weber M, Backsch C, et al. Establishment of a one-sided ex vivo human placenta perfusion model to assess adhesion and invasion behavior of T cell leukemia cell lines. Leuk Lymphoma 2013;54:1811-3. [Crossref] [PubMed]
- Nazarov I, Lee JW, Soupene E, et al. Multipotent stromal stem cells from human placenta demonstrate high therapeutic potential. Stem Cells Transl Med 2012;1:359-72. [Crossref] [PubMed]
- Schmidt A, Ladage D, Steingen C, et al. Mesenchymal stem cells transmigrate over the endothelial barrier. Eur J Cell Biol 2006;85:1179-88. [Crossref] [PubMed]
- Steingen C, Brenig F, Baumgartner L, et al. Characterization of key mechanisms in transmigration and invasion of mesenchymal stem cells. J Mol Cell Cardiol 2008;44:1072-84. [Crossref] [PubMed]
- Qin SQ, Kusuma GD, Al-Sowayan B, et al. Establishment and characterization of fetal and maternal mesenchymal stem/stromal cell lines from the human term placenta. Placenta 2016;39:134-46. [Crossref] [PubMed]
- Gahmberg CG, Nortamo P, Kantor C, et al. The pivotal role of the Leu-CAM and ICAM molecules in human leukocyte adhesion. Cell Differ Dev 1990;32:239-45. [Crossref] [PubMed]
- Quintavalla J, Uziel-Fusi S, Yin J, et al. Fluorescently labeled mesenchymal stem cells (MSCs) maintain multilineage potential and can be detected following implantation into articular cartilage defects. Biomaterials 2002;23:109-19. [Crossref] [PubMed]
- Li YJ, Xiao ZS, Peng CF, et al. Calcitonin gene-related peptide-induced preconditioning protects against ischemia-reperfusion injury in isolated rat hearts. Eur J Pharmacol 1996;311:163-7. [Crossref] [PubMed]
- Nitzsche F, Muller C, Lukomska B, et al. Concise Review: MSC Adhesion Cascade-Insights into Homing and Transendothelial Migration. Stem Cells 2017;35:1446-60. [Crossref] [PubMed]
- Castrechini NM, Murthi P, Gude NM, et al. Mesenchymal stem cells in human placental chorionic villi reside in a vascular Niche. Placenta 2010;31:203-12. [Crossref] [PubMed]
- Kusuma GD, Manuelpillai U, Abumaree MH, et al. Mesenchymal stem cells reside in a vascular niche in the decidua basalis and are absent in remodelled spiral arterioles. Placenta 2015;36:312-21. [Crossref] [PubMed]
- Ley K, Laudanna C, Cybulsky MI, et al. Getting to the site of inflammation: the leukocyte adhesion cascade updated. Nat Rev Immunol 2007;7:678-89. [Crossref] [PubMed]
- Barreiro O, Sanchez-Madrid F. Molecular basis of leukocyte-endothelium interactions during the inflammatory response. Rev Esp Cardiol 2009;62:552-62. [Crossref] [PubMed]
- Phillipson M, Heit B, Colarusso P, et al. Intraluminal crawling of neutrophils to emigration sites: a molecularly distinct process from adhesion in the recruitment cascade. J Exp Med 2006;203:2569-75. [Crossref] [PubMed]
- Ruster B, Gottig S, Ludwig RJ, et al. Mesenchymal stem cells display coordinated rolling and adhesion behavior on endothelial cells. Blood 2006;108:3938-44. [Crossref] [PubMed]
- Colucci M, Balconi G, Lorenzet R, et al. Cultured human endothelial cells generate tissue factor in response to endotoxin. J Clin Invest 1983;71:1893-6. [Crossref] [PubMed]
Cite this article as: Al-Sowayan B, Keogh RJ, Abumaree M, Georgiou HM, Kalionis B. An ex vivo human placental vessel perfusion method to study mesenchymal stem/stromal cell migration. Stem Cell Investig 2019;6:2.